Order Now
- Home
- About Us
-
Services
-
Assignment Writing
-
Academic Writing Services
- Urgent Assignment Help
- Writing Assignment for University
- College Assignment Help
- SPSS Assignment Help
- HND Assignment Help
- Architecture Assignment Help
- Total Assignment Help
- All Assignment Help
- My Assignment Help
- Student Assignment Help
- Instant Assignment Help
- Cheap Assignment Help
- Global Assignment Help
- Write My Assignment
- Do My Assignment
- Solve My Assignment
- Make My Assignment
- Pay for Assignment Help
-
Management
- Financial Management Assignment Help
- Business Management Assignment Help
- Management Assignment Help
- Project Management Assignment Help
- Supply Chain Management Assignment Help
- Operations Management Assignment Help
- Risk Management Assignment Help
- Strategic Management Assignment Help
- Logistics Management Assignment Help
- Global Business Strategy Assignment Help
- Consumer Behavior Assignment Help
- MBA Assignment Help
- Portfolio Management Assignment Help
- Change Management Assignment Help
- Hospitality Management Assignment Help
- Healthcare Management Assignment Help
- Investment Management Assignment Help
- Market Analysis Assignment Help
- Corporate Strategy Assignment Help
- Conflict Management Assignment Help
- Marketing Management Assignment Help
- Strategic Marketing Assignment Help
- CRM Assignment Help
- Marketing Research Assignment Help
- Human Resource Assignment Help
- Business Assignment Help
- Business Development Assignment Help
- Business Statistics Assignment Help
- Business Ethics Assignment Help
- 4p of Marketing Assignment Help
- Pricing Strategy Assignment Help
- Nursing
-
Finance
- Finance Assignment Help
- Do My Finance Assignment For Me
- Financial Accounting Assignment Help
- Behavioral Finance Assignment Help
- Finance Planning Assignment Help
- Personal Finance Assignment Help
- Financial Services Assignment Help
- Forex Assignment Help
- Financial Statement Analysis Assignment Help
- Capital Budgeting Assignment Help
- Financial Reporting Assignment Help
- International Finance Assignment Help
- Business Finance Assignment Help
- Corporate Finance Assignment Help
-
Accounting
- Accounting Assignment Help
- Managerial Accounting Assignment Help
- Taxation Accounting Assignment Help
- Perdisco Assignment Help
- Solve My Accounting Paper
- Business Accounting Assignment Help
- Cost Accounting Assignment Help
- Taxation Assignment Help
- Activity Based Accounting Assignment Help
- Tax Accounting Assignment Help
- Financial Accounting Theory Assignment Help
-
Computer Science and IT
- Robotics Assignment Help
- Operating System Assignment Help
- Data mining Assignment Help
- Computer Network Assignment Help
- Database Assignment Help
- IT Management Assignment Help
- Network Topology Assignment Help
- Data Structure Assignment Help
- Business Intelligence Assignment Help
- Data Flow Diagram Assignment Help
- UML Diagram Assignment Help
- R Studio Assignment Help
-
Law
- Law Assignment Help
- Business Law Assignment Help
- Contract Law Assignment Help
- Tort Law Assignment Help
- Social Media Law Assignment Help
- Criminal Law Assignment Help
- Employment Law Assignment Help
- Taxation Law Assignment Help
- Commercial Law Assignment Help
- Constitutional Law Assignment Help
- Corporate Governance Law Assignment Help
- Environmental Law Assignment Help
- Criminology Assignment Help
- Company Law Assignment Help
- Human Rights Law Assignment Help
- Evidence Law Assignment Help
- Administrative Law Assignment Help
- Enterprise Law Assignment Help
- Migration Law Assignment Help
- Communication Law Assignment Help
- Law and Ethics Assignment Help
- Consumer Law Assignment Help
- Science
- Biology
- Engineering
-
Humanities
- Humanities Assignment Help
- Sociology Assignment Help
- Philosophy Assignment Help
- English Assignment Help
- Geography Assignment Help
- Agroecology Assignment Help
- Psychology Assignment Help
- Social Science Assignment Help
- Public Relations Assignment Help
- Political Science Assignment Help
- Mass Communication Assignment Help
- History Assignment Help
- Cookery Assignment Help
- Auditing
- Mathematics
-
Economics
- Economics Assignment Help
- Managerial Economics Assignment Help
- Econometrics Assignment Help
- Microeconomics Assignment Help
- Business Economics Assignment Help
- Marketing Plan Assignment Help
- Demand Supply Assignment Help
- Comparative Analysis Assignment Help
- Health Economics Assignment Help
- Macroeconomics Assignment Help
- Political Economics Assignment Help
- International Economics Assignments Help
-
Academic Writing Services
-
Essay Writing
- Essay Help
- Essay Writing Help
- Essay Help Online
- Online Custom Essay Help
- Descriptive Essay Help
- Help With MBA Essays
- Essay Writing Service
- Essay Writer For Australia
- Essay Outline Help
- illustration Essay Help
- Response Essay Writing Help
- Professional Essay Writers
- Custom Essay Help
- English Essay Writing Help
- Essay Homework Help
- Literature Essay Help
- Scholarship Essay Help
- Research Essay Help
- History Essay Help
- MBA Essay Help
- Plagiarism Free Essays
- Writing Essay Papers
- Write My Essay Help
- Need Help Writing Essay
- Help Writing Scholarship Essay
- Help Writing a Narrative Essay
- Best Essay Writing Service Canada
-
Dissertation
- Biology Dissertation Help
- Academic Dissertation Help
- Nursing Dissertation Help
- Dissertation Help Online
- MATLAB Dissertation Help
- Doctoral Dissertation Help
- Geography Dissertation Help
- Architecture Dissertation Help
- Statistics Dissertation Help
- Sociology Dissertation Help
- English Dissertation Help
- Law Dissertation Help
- Dissertation Proofreading Services
- Cheap Dissertation Help
- Dissertation Writing Help
- Marketing Dissertation Help
- Programming
-
Case Study
- Write Case Study For Me
- Business Law Case Study Help
- Civil Law Case Study Help
- Marketing Case Study Help
- Nursing Case Study Help
- Case Study Writing Services
- History Case Study help
- Amazon Case Study Help
- Apple Case Study Help
- Case Study Assignment Help
- ZARA Case Study Assignment Help
- IKEA Case Study Assignment Help
- Zappos Case Study Assignment Help
- Tesla Case Study Assignment Help
- Flipkart Case Study Assignment Help
- Contract Law Case Study Assignments Help
- Business Ethics Case Study Assignment Help
- Nike SWOT Analysis Case Study Assignment Help
- Coursework
- Thesis Writing
- CDR
- Research
-
Assignment Writing
-
Resources
- Referencing Guidelines
-
Universities
-
Australia
- Asia Pacific International College Assignment Help
- Macquarie University Assignment Help
- Rhodes College Assignment Help
- APIC University Assignment Help
- Torrens University Assignment Help
- Kaplan University Assignment Help
- Holmes University Assignment Help
- Griffith University Assignment Help
- VIT University Assignment Help
- CQ University Assignment Help
-
Australia
- Experts
- Free Sample
- Testimonial
The Field of Nuclear Physics Assignment Sample
The branch of physics known as nuclear physics investigates the components and interactions of atomic nuclei. Nuclear physics has a variety of uses outside of nuclear power and nuclear weapons, including those in medicine (nuclear medicine, magnetic resonance imaging), materials engineering (ion implantation), archaeology, and other fields (radiocarbon dating).
Nuclear physics gave rise to particle physics, which was previously referred to under the same umbrella because of this.
The earliest proof that the atom had interior structure came from J. J. Thomson's discovery of the electron. J. J. Thomson's "plum pudding" concept, in which the atom was a huge positively charged ball with little negatively charged electrons lodged inside of it, was the recognised model of the atom at the turn of the 20th century. Alpha, beta, and gamma radiation are three different forms of radiation that are emitted by atoms that were discovered by physicists at the turn of the century. James Chadwick and Lise Meitner's experiments from 1911 and Otto Hahn's from 1914 both revealed that the beta decay spectrum was continuous rather than discrete. In other words, rather of the definite quantities of energies seen in gamma and alpha decays, electrons were released from the atom with a variety of energies. At the time, this presented a challenge for nuclear physics because it suggested that energy was not conserved in these decays.
Albert Einstein first proposed the concept of mass-energy equivalence in 1905. Although Becquerel, Pierre, and Marie Curie's research on radioactivity came before this, it wasn't until the nucleus's smaller subatomic particles, known as nucleons, were discovered that the source of radioactivity's energy could be identified.
Rutherford’s Team Discovers the Nucleus
"Radiation of the Particle from Radium in Passing Through Matter"[1] was published in 1907 by Ernest Rutherford. In a submission to the Royal Society[2], Geiger expanded on this work with experiments he and Rutherford had carried out, passing a particle through air, aluminium foil, and gold leaf. Geiger and Marsden published additional work in 1909[3], and in 1910 Geiger published significantly more material. [4] Rutherford presented the new theory of the atomic nucleus as we now understand it to the Royal Society in 1911–1912. He also explained the experiments at the time.
The crucial science assignment experiment that led to this discovery was carried out in 1909 by Ernest Rutherford's team, in which Hans Geiger and Ernest Marsden shot alpha particles (helium nuclei) at a thin layer of gold foil. According to the plum pudding model, the alpha particles should exit the foil with at most a little bending of their paths. Rutherford came up with the notion to tell his team to search for something that he was startled to see in action: a few particles were scattered at extreme angles, even going backwards in some cases. He compared it to shooting a bullet at tissue paper, which would cause it to deflect. The discovery eventually produced the Rutherford model of the atom, according to which an atom has a very small, extremely dense nucleus that contains the majority of its mass and is made up of heavy, positively charged particles with embedded electrons to balance the charge. This model was developed as a result of Rutherford's analysis of the data in 1911. (since the neutron was unknown). As an illustration, in this model (which is not the modern one), the nucleus of nitrogen-14 contained 21 particles total—14 protons, 7 electrons—and was encircled by an additional 7 electrons in orbit.
The Rutherford model performed admirably up until 1929, when Franco Rasetti conducted nuclear spin studies at the California Institute of Technology. In the Rutherford model of nitrogen-14, 20 of the total 21 nuclear particles were supposed to pair up to cancel each other's spins, and the final odd particle was supposed to leave the nucleus with a net spin of 1/2. By 1925, it was known that protons and electrons had a spin of 1/2. But Rasetti found that nitrogen-14 has a spin of 1.
James Chadwick Discovers the Neutron
In 1932, Chadwick discovered that radiation that Walther Bothe, Herbert L. Becker, Irene, and Fredric Joliot-Curie had detected was actually caused by a neutral particle he named the neutron, which has a mass similar to the proton (following a suggestion about the need for such a particle, by Rutherford). The same year, Dmitri Ivanenko proposed that neutrons are actually spin-1/2 particles, that the nucleus contains neutrons to account for the mass that cannot be attributed to protons, and that there are only protons and neutrons in the nucleus. The one unpaired proton and one unpaired neutron in this scenario each contribute a spin of 1/2 in the same direction, for a final total spin of 1, which directly solves the spin of nitrogen-14 problem.
With the discovery of the neutron, scientists were finally able to determine the percentage of binding energy present in each nucleus by comparing the nuclear mass to the mass of the protons and neutrons that made up the nucleus. When nuclear reactions were monitored and differences between nuclear masses were calculated in this manner, it was discovered that they agreed very closely (within 1% as of 1934) with Einstein's calculation of the equivalence of mass and energy.
Yukawa’s Meson Postulated to Bind Nuclei
To explain why the nucleus remains intact, Hideki Yukawa put forward the first significant hypothesis of the strong force in 1935. A hypothetical particle, later referred to as a meson, acted as a medium for a force between all nucleons, including protons and neutrons, in the Yukawa interaction. This force provided an explanation for why nuclei were resistant to proton repulsion and for why the attractive strong force had a smaller effective field than electromagnetic repulsion between protons. It was later discovered that the pi meson has the same characteristics as Yukawa's particle.
The contemporary atom model was finished with Yukawa's works. The strong nuclear force, unless it becomes too powerful, holds the tightly packed ball of neutrons and protons that makes up the centre of the atom together. Unstable nuclei can undergo beta decay, in which they eject an electron, or alpha decay, in which they emit an energetic helium nucleus (or positron). If the resultant nucleus is still excited after one of these decays, it may transition to its ground state by generating high-energy photons (gamma decay).
The study of the weak and strong nuclear forces—the latter of which Enrico Fermi explained in 1934 through the Fermi interaction—led scientists to collide nuclei and electrons at ever-increasing energy. The standard model of particle physics, which unifies the strong, weak, and electromagnetic forces, is the science of particle physics, which emerged from this research.
Modern Nuclear Physics
Main articles: Liquid-drop model and Shell model
Since heavy nuclei can have hundreds of nucleons, they can roughly be viewed as classical systems rather than quantum-mechanical ones. The nucleus in the resulting liquid-drop model has energy that comes from both surface tension and electrical repulsion of the protons. Numerous characteristics of nuclei, such as the overall trend of binding energy with respect to mass number and the nuclear fission event, can be replicated by the liquid-drop model.
However, quantum-mechanical effects are superimposed on this classical picture and can be explained by the nuclear shell model, which was largely created by Maria Goeppert-Mayer. Because their outer shells are filled, nuclei with specific numbers of neutrons and protons—the "magic numbers" 2, 8, 20, 50, 82, 126, etc.—are more stable than others.
There have also been other, more complex models for the nucleus put forth, such as the interacting boson model, which has pairs of neutrons and protons interact as bosons, similar to Cooper pairs of electrons.
The study of nuclei under extreme circumstances, such as high spin and excitation energy, is a significant portion of modern nuclear physics research. Additionally, high neutron-to-proton ratios or forms (like rugby balls) are possible for nuclei. Using ion beams from an accelerator and artificially produced fusion or nucleon transfer reactions, experimenters can produce such nuclei. There are indications that these experiments have led to a phase transition from normal nuclear matter to a new state, the quark-gluon plasma, in which the quarks mix with one another rather than being segregated in triplets as they are in neutrons and protons. Beams with even higher energies can be used to create nuclei at very high temperatures.
Modern Topics in Nuclear Physics
Spontaneous changes from one nuclide to another: nuclear decay
Main article: Radioactivity
There are around 256 stable isotopes total, and 80 elements have at least one of them. Stable isotopes are defined as those that have never been seen to degrade. Thousands more described isotopes exist, however they are unstable. These radioisotopes may be unstable and degrade across a wide range of timescales, from a few hundredths of a second to many billions of years.
For instance, a nucleus may be unstable and eventually decay if it has either too few or too many neutrons. For instance, within a few seconds of being generated, an oxygen-16 atom (8 protons, 8 neutrons) is transformed from a nitrogen-16 atom (7 protons, 9 neutrons) in a process known as beta decay. The weak nuclear force causes a neutron in the nitrogen nucleus to decay in this way, converting it into a proton, electron, and antineutrino. Because it now contains eight protons instead of the prior seven that made it nitrogen, the atom undergoes transmutation into another element (which makes it oxygen).
When a radioactive element undergoes alpha decay, a helium nucleus (2 protons and 2 neutrons) is released, along with another element and helium-4. The formation of a stable element frequently occurs after numerous phases of this kind, including other forms of decays.
A nucleus emits a gamma ray during gamma decay when it transitions from an excited state to a lower state. Then it becomes stable. The process has no impact on the element.
There may be further, stranger decays (see the main article). For instance, internal conversion decay, which produces high-speed electrons but is not beta decay and does not transform one element into another (unlike beta decay), uses the energy from an excited nucleus to eject one of the inner orbital electrons from the atom.
Nuclear Fusion
Main article: Nuclear fusion
It is conceivable for the strong force to fuse together two low mass nuclei when they are in extremely close proximity to one another. Nuclear fusion can only occur at extremely high temperatures or high densities because it requires a lot of energy to move the nuclei close enough together for the strong or nuclear forces to act. When two nuclei are sufficiently close to one another, the strong force overcomes their electromagnetic attraction and forces them to fuse together to form a new nucleus. Because the binding energy per nucleon rises with mass number up until nickel-62, the energy produced when light nuclei fuse together is extremely enormous. Four protons are fused into a helium nucleus, together with two positrons and two neutrinos, to create stars like our sun. The term "thermonuclear runaway" refers to the uncontrolled fusing of hydrogen into helium. Several research institutions are now working to find an economically feasible way to use the energy from a controlled fusion reaction (see JET and ITER).
The binding energy per nucleon for nuclei heavier than nickel-62 reduces as the mass number increases. Therefore, if a heavy nucleus splits into two lighter ones, energy could be released. Nuclear fission is the term for this atom-splitting process.
Alpha decay can be viewed as a particular variety of spontaneous nuclear fission. The four particles that make up the alpha particle are extremely closely connected to one another, making the creation of this nucleus in fission particularly likely. This mechanism results in a highly asymmetrical fission.
A self-igniting sort of neutron-initiated fission can be produced for some of the heaviest nuclei that emit neutrons during fission and that also readily absorb neutrons to initiate fission, in a process known as a chain reaction. (Chain reactions were recognised in chemistry before they were in physics, and many common events, such as fires and chemical explosions, are examples of them.) Nuclear power plants and fission-type nuclear weapons, like the two that the United States deployed against Hiroshima and Nagasaki at the conclusion of World War II, use the fission or "nuclear" chain-reaction to produce neutrons. While it is possible for heavy nuclei like uranium and thorium to spontaneously fission, this is significantly less likely than alpha decay.
There must be a critical mass of the element present in a certain location under specific circumstances for a neutron-initiated chain reaction to happen (these conditions slow and conserve neutrons for the reactions). There is only one known instance of a naturally occurring nuclear fission reactor, and it was operational over 1.5 billion years ago in two locations of Oklo, Gabon, Africa. Radioactive decay is responsible for almost half of the heat emitted from the Earth's core, according to measurements of natural neutrino emission. If any of this comes from fission chain reactions is unknown, though.
Production of Heavy Elements
The hypothesis holds that the existence of particles as we know them today was finally made feasible as the Universe cooled following the big bang. Protons (hydrogen) and electrons were the most prevalent particles produced in the big bang that are still readily observable to us today (in equal numbers). While some heavier elements were produced as a result of proton collisions, the majority of the heavy elements we observe today were produced in stars during a succession of fusion stages, including the proton-proton chain, the CNO cycle, and the triple-alpha process. During a star's evolution, heavier elements are produced one at a time. Energy is only released in fusion processes that take place below this threshold since the binding energy per nucleon peaks around iron. Nature uses the process of neutron capture because it is more energy efficient than fusion to produce heavier nuclei. The lack of charge in neutrons makes them easily absorbed by a nucleus. Either the rapid, or r process, or the slow neutron capture method—commonly referred to as the s process—is used to produce the heavy elements. The s process takes hundreds to thousands of years to achieve the heaviest elements of lead and bismuth in thermally pulsing stars (also known as AGB, or asymptotic giant branch stars). Because of the high temperature, high neutron flux, and ejected matter circumstances, it is believed that the r process occurs in supernova explosions. Particularly near the so-called waiting spots, which correspond to more stable nuclides with closed neutron shells, these stellar conditions cause the subsequent neutron captures to occur very quickly, involving particularly neutron-rich species that eventually beta-decay to heavier elements (magic numbers). The r procedure normally takes a few seconds to complete.
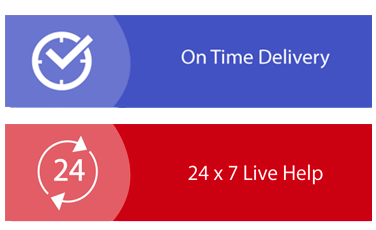
Download Samples PDF
Related Sample
- PRJM6010 Project and People Assignment 2
- Effect of Life Experiences on Psychology Theory Orientation
- 2128IBA The Management of Business Processes Assignment
- MBA642 Project Initiation Planning and Execution
- PROJ6002 Project Planning and Budgeting Assignment
- BIS1003 Introduction To Programming Assignment
- LC3002 English For Academic Purpose Resit Information
- CSIT985 Social Media for Organization Innovation Essay 2
- MIS604 Requirement Engineering Report
- COMP2003 Securing Networks Assignment
- MIS604 Requirement Engineering Case Study
- ENT201 Sales and Negotiation Strategies Assignment
- PROJ6003 Project Execution and Control Report
- COMP1702 Big Data Assignment
- MBA673 Business Analytics Lifecycle Report 3
- SITXSA002 Participate in Safe Food Handling Practices Instruction Assignment
- FPH201 First Peoples Culture History and Healthcare Report 1
- MGT502 Business Communication
- MKT60010 Marketing Management Assignment
- GDECE101 Early Childhood Care and Education
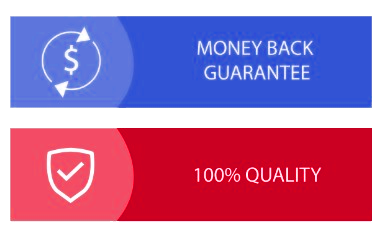
Assignment Services
-
Assignment Writing
-
Academic Writing Services
- Urgent Assignment Help
- Writing Assignment for University
- College Assignment Help
- SPSS Assignment Help
- HND Assignment Help
- Architecture Assignment Help
- Total Assignment Help
- All Assignment Help
- My Assignment Help
- Student Assignment Help
- Instant Assignment Help
- Cheap Assignment Help
- Global Assignment Help
- Write My Assignment
- Do My Assignment
- Solve My Assignment
- Make My Assignment
- Pay for Assignment Help
-
Management
- Financial Management Assignment Help
- Business Management Assignment Help
- Management Assignment Help
- Project Management Assignment Help
- Supply Chain Management Assignment Help
- Operations Management Assignment Help
- Risk Management Assignment Help
- Strategic Management Assignment Help
- Logistics Management Assignment Help
- Global Business Strategy Assignment Help
- Consumer Behavior Assignment Help
- MBA Assignment Help
- Portfolio Management Assignment Help
- Change Management Assignment Help
- Hospitality Management Assignment Help
- Healthcare Management Assignment Help
- Investment Management Assignment Help
- Market Analysis Assignment Help
- Corporate Strategy Assignment Help
- Conflict Management Assignment Help
- Marketing Management Assignment Help
- Strategic Marketing Assignment Help
- CRM Assignment Help
- Marketing Research Assignment Help
- Human Resource Assignment Help
- Business Assignment Help
- Business Development Assignment Help
- Business Statistics Assignment Help
- Business Ethics Assignment Help
- 4p of Marketing Assignment Help
- Pricing Strategy Assignment Help
- Nursing
-
Finance
- Finance Assignment Help
- Do My Finance Assignment For Me
- Financial Accounting Assignment Help
- Behavioral Finance Assignment Help
- Finance Planning Assignment Help
- Personal Finance Assignment Help
- Financial Services Assignment Help
- Forex Assignment Help
- Financial Statement Analysis Assignment Help
- Capital Budgeting Assignment Help
- Financial Reporting Assignment Help
- International Finance Assignment Help
- Business Finance Assignment Help
- Corporate Finance Assignment Help
-
Accounting
- Accounting Assignment Help
- Managerial Accounting Assignment Help
- Taxation Accounting Assignment Help
- Perdisco Assignment Help
- Solve My Accounting Paper
- Business Accounting Assignment Help
- Cost Accounting Assignment Help
- Taxation Assignment Help
- Activity Based Accounting Assignment Help
- Tax Accounting Assignment Help
- Financial Accounting Theory Assignment Help
-
Computer Science and IT
- Robotics Assignment Help
- Operating System Assignment Help
- Data mining Assignment Help
- Computer Network Assignment Help
- Database Assignment Help
- IT Management Assignment Help
- Network Topology Assignment Help
- Data Structure Assignment Help
- Business Intelligence Assignment Help
- Data Flow Diagram Assignment Help
- UML Diagram Assignment Help
- R Studio Assignment Help
-
Law
- Law Assignment Help
- Business Law Assignment Help
- Contract Law Assignment Help
- Tort Law Assignment Help
- Social Media Law Assignment Help
- Criminal Law Assignment Help
- Employment Law Assignment Help
- Taxation Law Assignment Help
- Commercial Law Assignment Help
- Constitutional Law Assignment Help
- Corporate Governance Law Assignment Help
- Environmental Law Assignment Help
- Criminology Assignment Help
- Company Law Assignment Help
- Human Rights Law Assignment Help
- Evidence Law Assignment Help
- Administrative Law Assignment Help
- Enterprise Law Assignment Help
- Migration Law Assignment Help
- Communication Law Assignment Help
- Law and Ethics Assignment Help
- Consumer Law Assignment Help
- Science
- Biology
- Engineering
-
Humanities
- Humanities Assignment Help
- Sociology Assignment Help
- Philosophy Assignment Help
- English Assignment Help
- Geography Assignment Help
- Agroecology Assignment Help
- Psychology Assignment Help
- Social Science Assignment Help
- Public Relations Assignment Help
- Political Science Assignment Help
- Mass Communication Assignment Help
- History Assignment Help
- Cookery Assignment Help
- Auditing
- Mathematics
-
Economics
- Economics Assignment Help
- Managerial Economics Assignment Help
- Econometrics Assignment Help
- Microeconomics Assignment Help
- Business Economics Assignment Help
- Marketing Plan Assignment Help
- Demand Supply Assignment Help
- Comparative Analysis Assignment Help
- Health Economics Assignment Help
- Macroeconomics Assignment Help
- Political Economics Assignment Help
- International Economics Assignments Help
-
Academic Writing Services
-
Essay Writing
- Essay Help
- Essay Writing Help
- Essay Help Online
- Online Custom Essay Help
- Descriptive Essay Help
- Help With MBA Essays
- Essay Writing Service
- Essay Writer For Australia
- Essay Outline Help
- illustration Essay Help
- Response Essay Writing Help
- Professional Essay Writers
- Custom Essay Help
- English Essay Writing Help
- Essay Homework Help
- Literature Essay Help
- Scholarship Essay Help
- Research Essay Help
- History Essay Help
- MBA Essay Help
- Plagiarism Free Essays
- Writing Essay Papers
- Write My Essay Help
- Need Help Writing Essay
- Help Writing Scholarship Essay
- Help Writing a Narrative Essay
- Best Essay Writing Service Canada
-
Dissertation
- Biology Dissertation Help
- Academic Dissertation Help
- Nursing Dissertation Help
- Dissertation Help Online
- MATLAB Dissertation Help
- Doctoral Dissertation Help
- Geography Dissertation Help
- Architecture Dissertation Help
- Statistics Dissertation Help
- Sociology Dissertation Help
- English Dissertation Help
- Law Dissertation Help
- Dissertation Proofreading Services
- Cheap Dissertation Help
- Dissertation Writing Help
- Marketing Dissertation Help
- Programming
-
Case Study
- Write Case Study For Me
- Business Law Case Study Help
- Civil Law Case Study Help
- Marketing Case Study Help
- Nursing Case Study Help
- Case Study Writing Services
- History Case Study help
- Amazon Case Study Help
- Apple Case Study Help
- Case Study Assignment Help
- ZARA Case Study Assignment Help
- IKEA Case Study Assignment Help
- Zappos Case Study Assignment Help
- Tesla Case Study Assignment Help
- Flipkart Case Study Assignment Help
- Contract Law Case Study Assignments Help
- Business Ethics Case Study Assignment Help
- Nike SWOT Analysis Case Study Assignment Help
- Coursework
- Thesis Writing
- CDR
- Research